A bright future for light microscopy Understand article
Want to catch an enzyme in the act? Or watch an embryonic brain hard-wire itself? Russ Hodge from the European Molecular Biology Laboratory in Heidelberg, Germany, explains how recent developments in microscopy show cells and organisms at work.
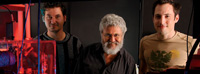
Bastiaens, Eric Karsenti and
Philipp Niethammer
Between the 1830s and 1870s, a series of improvements in light microscopes led to a new era in biology. Earlier microscopes suffered from poor resolution and image distortion because they relied on a single lens. The addition of a second lens solved part of this problem, and in 1830, English opticist Joseph Jackson Lister discovered that setting the two lenses a specific distance apart eliminates a type of distortion called spherical aberration. The result was a huge jump in resolution that permitted German physiologist Theodor Schwann and Matthias Jacob Schleiden, a German botanist, to prove that animal tissues were composed of cells.
In the molecular age, the focus of most biologists has moved to proteins and other molecules that are too small to observe individually with the light microscope. Under the electron microscope, very large molecules or complexes can sometimes be seen as fuzzy shapes, but the most important details are still lacking, and it is impossible to use this method on living cells or tissues.
In the mid-1990s, scientists discovered that a molecule from jellyfish called green fluorescent protein, which makes the animal fluoresce, could be attached to specific proteins in other organisms. This process of tagging created new, glowing molecules that could be tracked under the light microscope as they moved through living cells and tissues. It gave researchers a fairly simple way to obtain information about when and where specific proteins are produced in the body, where they end up in cells, and what they might be doing there.
Now biologists and physicists have developed methods to obtain even more information from fluorescent proteins. And another invention is permitting researchers to watch biological processes as they happen, in three dimensions. Scientists at the European Molecular Biology Laboratory (EMBL) in Heidelberg, Germany, are using these techniques to take a new look at some of the most fascinating questions in biology.
FRET, FRAP and FLIM
Philippe Bastiaens’ microscope looks like a cross between a kitchen counter and a laser light show. The instrument is mounted on a metal table large enough to seat ten people. When the young Dutch scientist flips a switch, a needle-thin ray of blue light zips between mirrors and lenses in a zigzag pattern.
The job of the laser is to illuminate a sample that contains fluorescent proteins. It excites the atoms of the tagged molecule, which then releases energy and makes the molecule visible. This happens only once before the molecule’s fluorescence is spent. If a large number of identical, tagged molecules are excited, they emit a unique pattern of energy. Philippe’s instruments allow him to measure this energy with extreme precision, which can yield important information about a protein’s activity.
“For example, the pattern of emission changes when the protein binds to another molecule,” Philippe says. “So we can detect the rates at which proteins bind and release each other. These are often reactions that happen so fast you can’t detect them any other way.” The technique is called FLIM, for fluorescence lifetime imaging microscopy.
“There are other differences that can be detected as well,” Philippe says. “Suppose that the protein you have tagged interacts with another molecule and causes a chemical change in it. When this happens, you find yet another fluorescence pattern. You can make profiles for all these different things and tell which one is happening. It puts us in the position of being able not only to watch close encounters between molecules but also to observe what they are doing to each other. Previously, if you wanted to say that one molecule is activating another, you had to rely on much less direct evidence.”
If you illuminate a region of a sample for too long (for example, a section of the cell nucleus, or another cellular compartment), all of the fluorescent energy is used up, and the region becomes bleached. Philippe and his colleagues have used this bleaching technique to watch how molecules move through the cell. For example, receptor proteins on the outer membrane help cells receive signals from their neighbours and the environment. Scientists believed that these molecules are brought inside the cell for recycling, but it has been difficult to prove. They could see receptor proteins in the cell interior, but it was hard to tell whether these molecules were travelling towards the membrane or had been brought back in.
Philippe and his colleagues focused the laser to bleach a tiny region inside the cell where receptor proteins are known to collect. Then they blocked the production of new proteins, to stop the flow of outward-moving molecules. When the bleached area once again filled with fluorescent proteins, the scientists knew that they were being brought in from the membrane. This method is called FRAP, for fluorescence recovery after photobleaching.
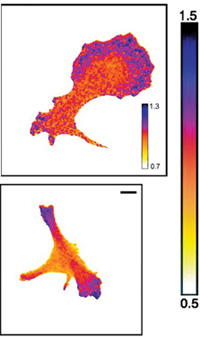
cells is the result of
interactions between
molecules. The cell at the top
is at rest; the cell at the
bottom is crawling along a
surface. Using FRET,
scientists can reveal where
particular proteins are
binding to each other,
explaining changes in the
cell’s structure and
behaviour. Blue shows areas
where many stathmin
proteins are binding to
molecules called tubulin. This
is happening at a much lower
rate in the red areas
Over the past decade, scientists have added red, yellow and new shades of green to the palette of fluorescent protein tags. One of the most important new techniques in microscopy involves watching the behaviour of two molecules with tags of different colours. Philippe compares FRET (fluorescence resonance energy transfer) to the behaviour of a bucket of sand.
“If two molecules approach each other so closely that they are physically interacting with each other, you detect changes in the fluorescence that they emit,” he says. “Suppose that you punch a hole in your bucket; the sand runs out at a certain rate. If you punch a second hole, the rate at which the bucket empties is different. That’s a bit like what happens in FRET. The second fluorescent molecule absorbs some of the energy emitted by the first. By detecting the difference in the emission of fluorescence, you observe something that scientists have been trying to see for a long time – direct interactions between the proteins.”
Philippe’s lab has used FRET to solve some crucial questions. How many receptor proteins are needed to receive a signal, and what happens when they do? One study showed that for a particular receptor, only a tiny number of molecules needed to be activated to set off a rapid chain reaction of chemical signalling within the cell. (Only a small point on the cell surface flashed the fluorescent signature that marked the activation of a receptor.) And the group’s most recent work shows how concentrations of proteins within particular regions of the cell help create structures called spindles that are necessary for cell division. “Scientists have believed that different chemical sub-environments in the cell play a role in structuring it,” Philippe says. “But it’s been hard to prove. That’s one of the things that FRET is helping us do, by painting a picture of the concentrations of different molecules as they interact with each other.”
SPIM
Ernst Stelzer’s microscopy group at EMBL has invented or helped improve many types of microscopes. Their latest instrument, called the selective plane illumination microscope (SPIM), allows scientists to peer directly into the bodies of living organisms as they develop.
Ernst and his colleagues had already built confocal microscopes that use a laser to scan a sample layer-by-layer at an amazing speed; a computer then assembles the image slices into a three-dimensional stack. “That doesn’t go far enough,” he says, “because it’s still fairly restricted to looking at tissues on flat surfaces – not the real context of cells and organisms.” So he began looking for a way to scan whole organisms in their three-dimensional environments.
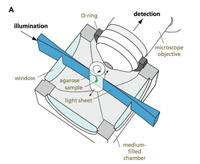
SPIM
The first innovation was to put the sample – such as an embryonic fish – into a liquid tube, so that it could be seen from all sides. But this didn’t solve a crucial problem of resolution. Most light microscopes illuminate a sample along the optical axis – shining a light from the position of the detector (an eye, or the camera) and looking at a reflection, or shining a light through the sample in the direction of the detector.
SPIM, on the other hand, passes a very thin sheet of light through the specimen from the side. Only the objects in that slice – such as fluorescent proteins – are illuminated. The tube is quickly rotated so that images can be captured from different angles. As with the confocal microscope, a computer assembles the slices into three-dimensional images – this time a smoothly running film.
“The advantage of bringing in light from the side has a lot to do with how microscopes achieve resolution,” says Ernst. “If you shine light at an object and view it along the same direction, there are situations where there is just no simple way to judge what is in front and what is behind, or exactly how far away it is. You can obtain good resolution along the two-dimensional surface facing you, and you can study different layers of a sample by moving the focal plane up and down, but a simple fact is that you are still illuminating objects above and below the focal plane, which distorts the two-dimensional image. They are inevitably fuzzy – extended in the third dimension.”
Images from SPIM are so sharp that it is possible to observe extremely subtle patterns within tissues. For example, developmental biologists hope to capture momentary bursts of genetic activity that have an important role in creating structures in the body, but which happen so fast that they are rarely observed.
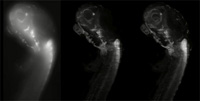
standard confocal
microscope. Compare with
the images of the same type
of specimen taken with SPIM
(centre and right)
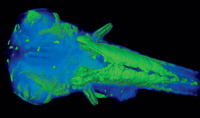
reconstruction of proteins
in the fish
The most immediate application is the chance to watch the development and physiology of inner organs. Jochen Wittbrodt’s lab, for example, is studying the development of a small fish called medaka. One project is to look at the heart, and with SPIM, an unprecedented view of its development in space and time can be captured. The scientists can visualise an organism’s first heartbeats – by peering straight through the body into muscle tissues. They are also getting an entirely new perspective on things that have been studied for a long time, such as the way the retina rounds up to form the eye cup. By watching how this works in both healthy fish and those with mutations, they have discovered that the textbook version – obtained from static pictures – has to be changed.
Another project with clear medical applications helped attract the interest of Zeiss, one of the world’s major manufacturers of microscopes, with plans to further develop and market the instrument. “Fish have an amazing ability to regenerate damaged nerve pathways, and we can watch in real time as their axons re-establish contact,” says Jochen. “Thus the new microscope has become a screening tool to help us find the genes that affect this process. One day that information may help us reawaken this regenerative capacity in our own tissues.”
Institutions
Review
There have been significant advances in microscopy in the last decade or so, particularly with the introduction of confocal microscopy. This interesting and informative article describes new microscopy techniques – fluorescence resonance energy transfer (FRET), fluorescence recovery after photobleaching (FRAP), and fluorescence lifetime imaging microscopy (FLIM) – and their fascinating applications. Green fluorescent protein, a molecule that fluoresces, is used to tag proteins so that molecular behaviour, for example receptor protein interaction and spindle structure and synthesis in cell division, can be visualised. Further development of the confocal microscope has resulted in the selective plane illumination microscope (SPIM), which can scan whole, untreated tissue using a laser to take image slices which are reassembled by a computer programme to form a three-dimensional image. This has been used to track the development of the eye cup, resulting in new information that overturns the current model.
Advanced-level biology students and their teachers usually consider the application of light and electron microscopy, discussing their limitations. This article will inform them of the fascinating developments that take these techniques further and help scientists discover mechanisms in the hidden world of molecular interaction. The article is well written, and is enhanced with illustrations that bring it to life for both teachers and students.
Shelley Goodman, UK