Looking to the heavens: climate change experiments Teach article
In the second of two articles, Dudley Shallcross, Tim Harrison, Steve Henshaw and Linda Sellou offer chemistry and physics experiments to harness the Sun’s energy and measure carbon dioxide levels.
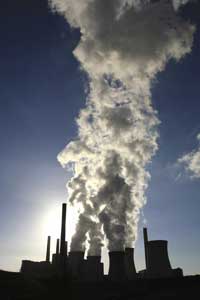
Discussions of climate change in the science classroom can be very wide-ranging, but different sources of energy and their consequences will probably have a role. The topics raised are likely to include different fuels that can be used, how effective they are and how they are produced; alternatives to combustion; solar energy; and the importance of carbon dioxide in global warming. Below, we suggest two laboratory activities to support physics and chemistry lessons on climate change. Three activities relating to fuels were published in Shallcross et al (2009).
1) Grätzel cells: energy from sunlight
The Sun, of course, is the source of most used energy on Earth, besides geothermal and nuclear energy – including that released from fossil fuels or modern ‘green’ fuels. But sunlight can also be used directly as a source of energy, as can be demonstrated in the classroom using Grätzel cells, also called ‘nanocrystalline dye solar cells’ or ‘organic solar cells’. Named after their inventor, the Swiss engineer Michael Grätzel, Grätzel cells convert sunlight directly into electricity by artificial photosynthesis using natural dyes found, for example, in cherries, blackberries, raspberries and blackcurrants. These purple-red dyes, known as anthocyaninsw1, are very easy for school students to extract from fruits and leaves by simply boiling them in a small volume of water and filtering.
These cells are very promising because they are made of low-cost materials and do not need elaborate apparatus to manufacture. Although their conversion efficiency is less than that of the best thin-film cells, their price/performance ratio (kWh/M2/annum) is high enough to allow them to compete with electricity generation from fossil fuels. Commercial applications, which were held up due to chemical stability problems, are now forecast in the European Union Photovoltaic Roadmapw2 to be a potentially significant contributor to renewable electricity generation by 2020.
Grätzel cells separate the two functions provided by silicon in a traditional cell design: normally, the silicon acts as the source of photoelectrons, as well as providing the electric field to separate the charges and create a current. In the Grätzel cell, the bulk of the semiconductor is used solely for charge transport, while the photoelectrons are provided from a separate photosensitive dye (the anthocyanin). Charge separation occurs at the surfaces between the dye, semiconductor and electrolyte.
The dye molecules are quite small (at the nanometre scale), so to capture a reasonable amount of the incoming light, the layer of dye molecules needs to be fairly thick – much thicker than the molecules themselves. To address this problem, a nanomaterial is used as a scaffold to hold large numbers of the dye molecules in a 3D matrix, increasing the number of molecules for any given surface area of the cell. In existing designs, this scaffolding is provided by the semiconductor material (titanium oxide), which serves double duty.
Grätzel cells can be made from scratch, but getting hold of the pre-treated glass that makes one side conductible is not easy. Moreover, baking the titanium dioxide paste into the glass surface requires the use of a furnace for about 24 hours. Therefore, it is easier to use commercial kits, such as those available from the Dutch company Mansolarw3, which allow six Grätzel cells to be assembled per set, costing approximately 80 Euros. If you already have some experience using the required equipment and prefer to build your own Grätzel cells, however, you will find an outline of the required steps below:
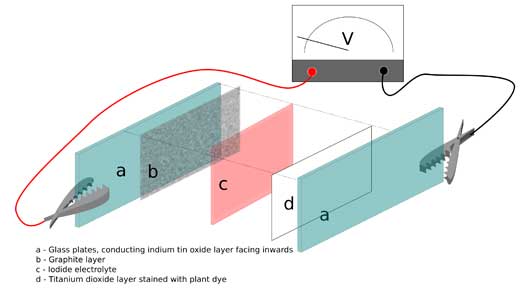
Image courtesy of Marcus Medley, Bristol ChemLabS
- Take two glass plates, each about the size of a microscope slide, one side of which has been treated with indium tin oxide to render it electroconductible.
- One plate needs to have titanium dioxide baked onto its uncoated side. The titanium dioxide forms a highly porous structure with a very high surface area, to which the dye can bind. A word of caution: it is very easy to scratch the titanium dioxide powder off the glass plate, and whilst you can purchase titanium dioxide paste, it is inconvenient to bake the plates for a considerable time with a new coating in a furnace. Storage is therefore a consideration. The easiest solution is to purchase the materials ready-made.
- Cover the other plate with a layer of pencil graphite by simply rubbing a pencil over the uncoated surface of the glass.
- Fill a Petri dish with anthocyanin dye. Soak the photosensitive anthocyanin dye onto the titanium dioxide by placing the corresponding plate in the Petri dish, then dry with a hair drier. The dye is left covalently bonded to the surface of the titanium dioxide. After use, it is easy to remove the old anthocyanin pigments using ethanol or propanone (acetone).
- Assemble the cell as follows from bottom to top:
- At the bottom will be the graphite plate, graphite side up, serving as a cathode.
- Use a solution of iodine dissolved in potassium iodide as an electrolyte and squirt between the plates.
- On top will be the dye-coated titanium dioxide plate, indium tin oxide side up. The indium tin oxide will serve as transparent anode.
- Use a paper clip to keep the plates together.
- Use two crocodile clips to clip the overlapping pieces of glass (top and bottom, see image) of the Grätzel cell and connect with two electrical leads to a multimeter to take readings.
- Shine light onto the cell. If investigating this apparatus in northern Europe during the winter months, a microscope lamp or a desk lamp will be useful to provide the light.
- Use a light meter to determine the light intensity falling on the Grätzel cells.
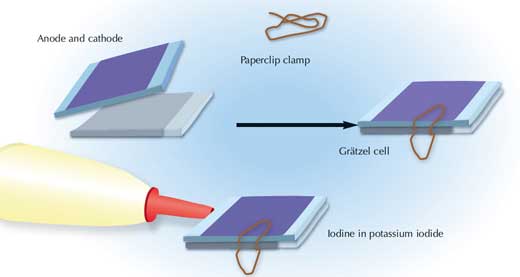
Image courtesy of Nicola Graf
For amusement, the Grätzel cells can be used to power different mechanisms. For example, you can replace the batteries in a calculator with leads that allow several small Grätzel cells in series to power it. Alternatively, you can also power the music circuits from birthday greetings cards or small motors with the cells.
Students may carry out a number of investigations with these cells. These include how the current or voltage produced varies with:
- Anthocyanins from different sources
- Plant pigments other than anthocyanins
- The concentration of the anthocyanin solution used
- The area of the titanium dioxide layer exposed to anthocyanins
- The temperature of the cells
- The frequency of light (using coloured filters)
- Light intensity (using a microscope lamp as a light source)
- Several cells in series or in parallel.
Details on the chemistry behind these cells can be found in an online articlew4.
2) Detecting atmospheric carbon dioxide levels
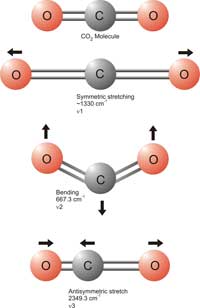
Click to enlarge image
Image courtesy of Marcus Medley,
Bristol ChemLabS
CO2 is the most commonly known greenhouse gas and one of the major concerns in discussions of climate change. One might well ask how levels of CO2 are measured in air samples, particularly as their concentrations are so low: the answer is infra-red spectroscopy. Carbon dioxide molecules absorb specific frequencies of infra-red radiation, which affect the covalent bonds between the carbon and oxygen atoms, depending on the energy. Low energies cause a bond-bending motion, and high energies cause bond stretching. The frequencies at which this occurs are within the infra-red part of the electromagnetic spectrum (between 4000 and 650 wavenumbers). A wavenumber is the reciprocal of wavelength and is a unit commonly used in infra-red spectroscopy. This effect can be used to determine the CO2 concentration as follows.
There are two main types of carbon dioxide sensor (see Harrison et al, 2006). The more expensive research sensors pump air through the sensor, whereas the cheaper devices rely on the diffusion of air. Air passes into an absorption cell, which is effectively a small darkened cylinder within the sensor.
At one end of the absorption cell, there is an infra-red light source coupled to a fixed wavelength filter, so as to provide a narrow band source of infra-red light around 2350 cm-1 (wavenumbers). At the other end of the tube, there is an infra-red detector or photon counter that measures the infra-red light intensity. The more CO2 molecules in the air sample, the more infra-red radiation is absorbed in the cell, and the less infra-red radiation reaches the detector. For small absorptions, the Beer-Lambert law tells us that
Concentration = (1-(I/I0)) / σl
where:
- l is the path length (length of the cell)
- σ is the absorption cross-section for CO2 at the wavelength being used and is known to a high accuracy
- (I/I0) is the ratio of infra-red radiation arriving at the detector when it has an air sample in it (I) to when the cell is empty (I0)
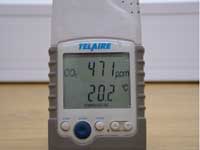
dioxide sensor
Image courtesy of Bristol ChemLabS
I0 is not measured for each reading, but will be measured frequently to check that there are no appreciable fluctuations in the instrument’s infra-red light intensity.
Students who have used such sensors, on loan from the University of Bristol, have been surprised that the measured CO2 level inside an empty classroom is much greater than that outside, well above 0.037% (0.037/100 x 1 x 106 = 370 ppm) reported for the CO2 atmospheric concentration in some textbooks. New school buildings in the UK appear to have windows that are not designed to be opened, so the exhaled CO2 accumulates!
The CO2 sensors that we use with students are tuned to the CO2 ν3 asymmetric bond stretch at 2349 wavenumbers (Harrison et al, 2006). An asymmetric stretch is where the double bonds between carbon and oxygen (C=O) absorb energy, and one of the two bonds lengthens while the other one contracts (see diagram). For CO2 there can only be one asymmetric stretch. This particular bond stretch is important because carbon dioxide is the only molecule present in high quantities in the atmosphere to absorb at 2349 wavenumbers. Therefore, only absorption by CO2 can cause a change in infra-red light intensity at this wavelength.
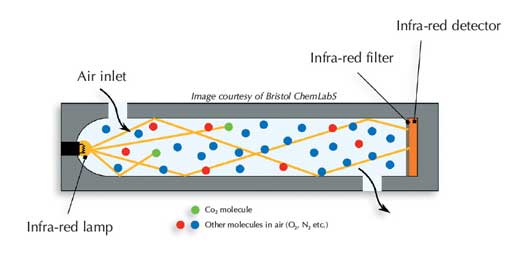
Image courtesy of Bristol ChemLabS
Bristol ChemLabS would be interested to hear from schools across Europe that would like to borrow one of these easy-to-use meters for research into the carbon dioxide concentration of air samples. Although the instruments are commercially available, they are quite expensive and thus not commonly available in schools or colleges.
References
- Harrison T, Shallcross D, Henshaw S (2006) Detecting CO2 – the hunt for greenhouse-gas emissions. Chemistry Review 15: 27-30
- Shallcross D, Harrison T (2008a) Climate change modelling in the classroom. Science in School 9: 28-33.
- Shallcross D, Harrison T (2008b) Practical demonstrations to augment climate change lessons. Science in School 10: 46-50.
- Shallcross D, Harrison T, Henshaw S, Sellou L (2009) Fuelling interest: climate change experiments. Science in School 11: 38-43.
Web References
- w1 – For more information on anthocyanins and their natural functions, see Wikipedia: http://en.wikipedia.org/wiki/Anthocyanin
- w2 – The 2002 European Union Photovoltaic Roadmap can be downloaded from the PV-NET website (http://paris.fe.uni-lj.si/pvnet) or here: http://tinyurl.com/n8cwfv
- w3 – A supplier for Grätzel cell kits is the Dutch company Mansolar: www.mansolar.com
- w4 – To find out more about the chemistry behind Grätzel cells, see the Royal Society of Chemistry website (www.rsc.org) or here: http://tinyurl.com/mr3bec
Resources
- SchoolCO2Web offers information and teaching material for measuring and sharing carbon dioxide data across schools in Europe: http://fwn-school-co2-net.hosting.rug.nl
Review
The article is a good complement to the previous trilogy of climate change articles by the same authors, on modelling climate (Shallcross et al, 2008a) and classroom experiments (Shallcross et al, 2008b, 2009). This time, the authors highlight the technological side of the climate change issue. They propose a hands-on activity as a possible alternative to conventional solar cells and an investigation of the CO2 content in different environments using professional equipment that they offer to lend to schools.
I recommend this set of articles to secondary-school science teachers looking for a full set of didactical materials to address the complex topic of climate change, global warming and energy resources. The style is plain enough for non-native English speakers, and the web references allow further learning on the subject. The article is also a valuable starting point for planning a stimulating interdisciplinary science curriculum.
Possible comprehension questions include:
- Which of the following statements about anthocyanins is true?
- they can perform artificial photosynthesis
- they perform photosynthesis in plants
- they are used in thin-film cells
- they are chemically stable
- Which of the following statements about titanium dioxide is false?
- it acts as a semiconductor
- it provides a 3D scaffold for the dye molecules
- it has to be baked on the glass surface
- it is electro-conductible
- Carbon dioxide sensors can measure
- the greenhouse effect
- infra-red light absorption
- air pollution
- UV light absorption
Giulia Realdon, Italy