Photoacoustics: seeing with sound Understand article
An advanced technology that combines high-frequency sound waves with laser light is giving researchers and clinicians a new way of seeing living tissue.
The saying ‘seeing is believing’ is sometimes as true in science as in other areas of life. Scientific images open up hidden vistas that are invisible to our unaided eyes, from the microscopic world of cells, molecules and even atoms to the most distant galaxies. Many of these images are made using systems that rely on light waves. But in biology and medicine, such systems have some drawbacks: light can’t travel far through biological tissue without being scattered, blurring the resulting images and preventing us from seeing deep inside the body (Ntziachristos, 2010). While X-rays can penetrate further, they are harmful to cells, so their use has to be kept to a minimum.
To overcome these problems, other imaging methods have been developed that rely not on light but on other kinds of wave – such as acoustic (sound) waves, which are used in the familiar technology of ultrasound imaging. Thanks to their longer wavelengths, acoustic waves are scattered much less easily by biological tissues and can penetrate further, allowing clinicians to monitor babies developing in the womb, to study blood flowing through veins, and to identify defects in the heart. However, standard ultrasound images generally have poor resolution, and they cannot compete with the clarity that light and X-ray images allow.
However, there is now an imaging technology that combines light with sound and promises the advantages of both. Aptly named ‘photoacoustic imaging’, this technique is based on the photoacoustic effect discovered by Scottish-born inventor Alexander Graham Bell in 1880.
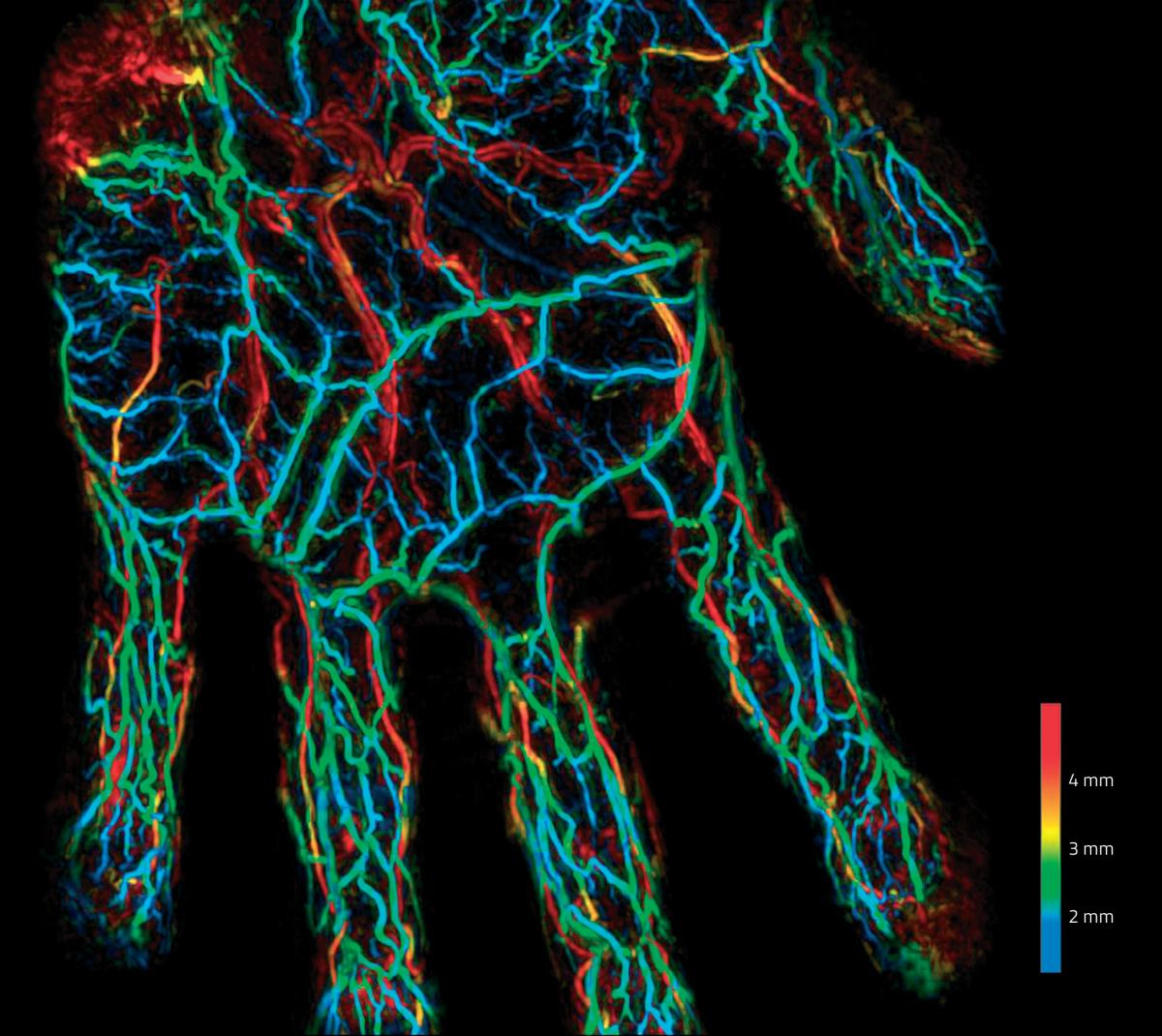
Matsumoto et al. (2018) Scientific Reports, CC BY 4.0
How photoacoustic imaging works
As Bell discovered more than a century ago, certain materials emit sound waves when struck by rapidly pulsing light. To understand why, it helps to think of the process as two consecutive steps.
In the first step, the absorption of light generates heat – an effect that will be familiar to anyone who has left a car parked without shade on a sunny day. The light energy causes molecules in the exposed material to enter a high-energy, ‘excited’ state. This state is not stable: within a trillionth or so of a second, the molecules revert to their former stable state by emitting heat energy. The same process happens when we shine laser light onto a cell: certain molecules called chromophores, or pigments, absorb the light energy and then heat up.
In the second step, the heat generates pressure changes – because, when an object is heated, it expands. If this happens to light-absorbing pigments inside a cell, the pigment molecules start to vibrate strongly and push on the surrounding structures, causing local changes in pressure. A single expansion is not sufficient to generate sound waves, however. For that to happen, the pressure must oscillate rapidly – which is exactly what occurs if the laser is made to fire a succession of very rapid pulses, each lasting a billionth of a second. The resulting fleeting expansions, alternating with cooling and contraction, generate a high-pitched sound wave with a frequency of 1–100 MHz – about 50–5000 times higher than the highest frequency our ears can detect. This sound wave can then be detected and used to produce clear, high-resolution images of biological structures, including those normally hidden beneath other tissues (figure 1).
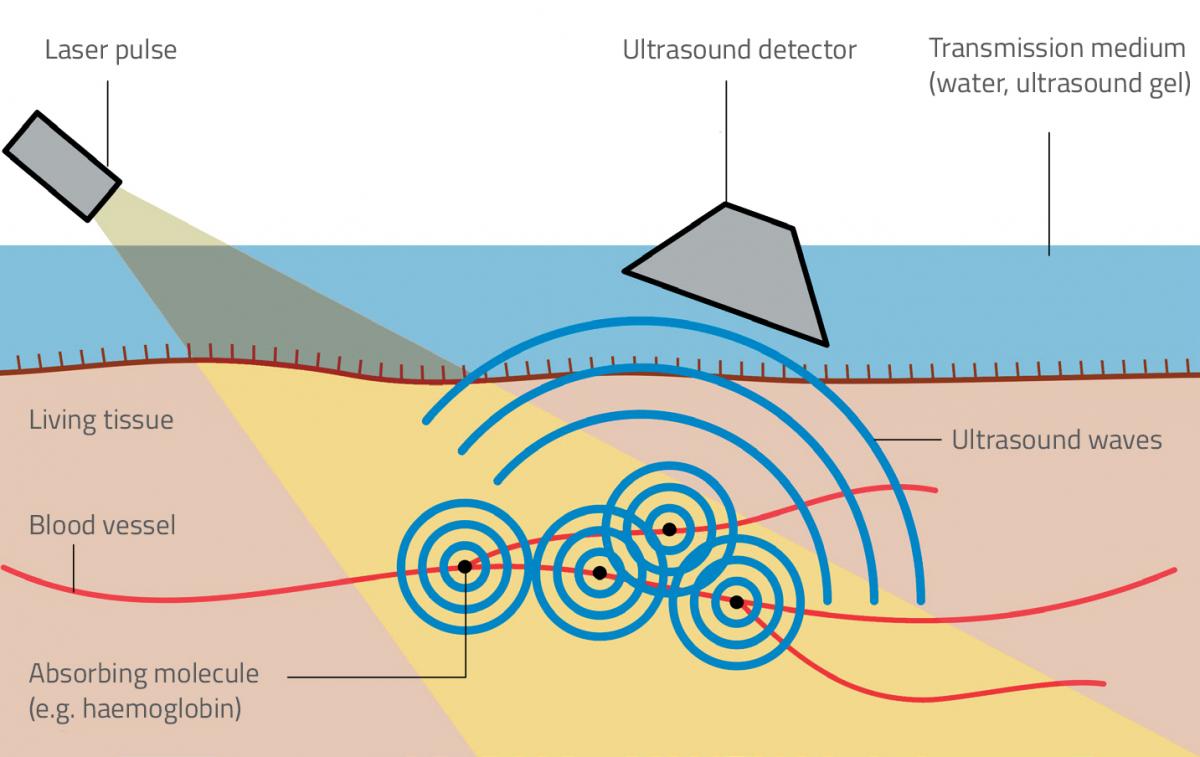
Jakub Czuchnowski
Using photoacoustic imaging
The photoacoustic approach has distinct advantages over the standard imaging technique, known as fluorescence microscopy. Here, a fluorescent marker is added to a sample and then made to fluoresce (re-emit light) by a light beam of a controlled wavelength. In photoacoustic microscopy, no additional ‘marker’ molecules need to be added, and the technique allows all absorbing molecules – including those naturally present in living organisms – to be imaged. In addition, particular types of molecule can be revealed selectively by changing the wavelength of the excitation light, and using multiple wavelengths allows us to see different tissue components at the same time.
Photoacoustic imaging is also being developed to create a new form of tomography – a computer technology whereby 3D images are built up by integrating digital information. Photoacoustic tomography relies on the fact that each absorbing molecule emits a spherical pressure wave that propagates in three dimensions. Detectors placed in different locations pick up the incoming pulses at slightly different times, and the software uses the time differences to calculate the point from which the spherical waves originated (Ntziachristos et al., 2005).
In practice, pressure waves originating from single molecules are too weak to be observed. In photoacoustic tomography, we typically detect signals coming from clusters of billions or trillions of molecules, which correspond to clumps of 10–1000 cells. For comparison, in clinical MRI (a widely used form of tomography), the resolution is on the order of 1 mm3, which corresponds to around one million cells.
Medical applications
Photoacoustic imaging has great potential in medicine, where scientific imaging is an important and powerful diagnostic tool. It is especially well suited to imaging structures that contain blood, since haemoglobin – the red, oxygen-carrying pigment in blood cells – shows up strongly in photoacoustic images. One application lies in tumour detection (figure 2). When tumours are growing, they trigger the reorganisation of surrounding blood vessels, often causing many new blood vessels to form in a process called neovascularisation. These blood vessels can be seen clearly by tuning the laser to the absorption frequency of the haemoglobin. Preliminary studies suggest that using photoacoustic imaging to reveal neovascularisation might be more effective at detecting breast cancer than ultrasound mammography (Heijblom et al., 2016). And, unlike X-rays, CT scanning and PET scanning, photoacoustic imaging does not expose patients to potentially harmful radiation.
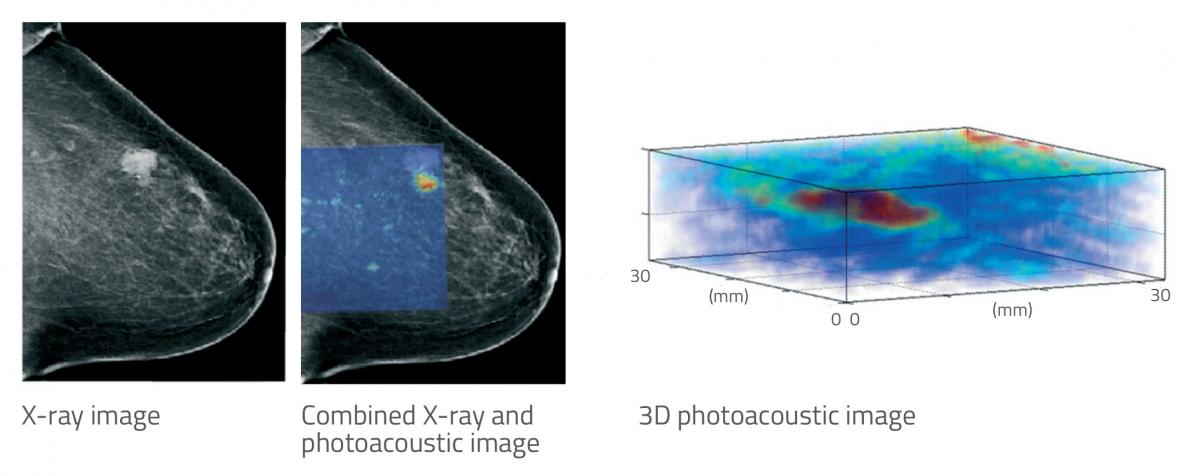
Heijblom et al. (2016) European Radiology, CC BY-NC 4.0
Another application is in detecting arteriosclerosis – the thickening and hardening of arteries that is often a precursor to heart disease or stroke. For this, imaging has to be done through an endoscope equipped with an optical fibre. The fatty ‘plaque’ that builds up inside arterial walls in arteriosclerosis is easy to distinguish from normal tissue, as it has a different absorption spectrum. Again, preliminary research has shown the promise of more accurate diagnosis.
Research applications
Some scientific challenges are best tackled by using ‘model organisms’ such as mice and rats, rather than by studying the human body directly. Rodents are important model organisms in cancer biology and also neuroscience, and their small size makes imaging their internal anatomy relatively easy because the light does not need to penetrate very far. A number of cancer studies involving photoacoustics have already been carried out with mice: for example, tracking the growth and progression of tumours (Jathoul et al., 2015).
Using rodents as a model also allows us to investigate how the mammalian brain works. There are around 80 billion neurons in the human brain, but the mouse brain has only around 70 million, making it much easier to image in detail. Until now, studies of the rodent brain have relied on imaging technologies that reveal different parts of a brain ‘lighting up’ as they function. Typical experiments involve stimulating a mouse with smells, sounds or visual stimuli and then imaging the whole brain to see where the sensory information is processed. The standard imaging technology used for this is functional MRI (fMRI). However, as well as having rather poor spatial resolution, fMRI does not reveal directly which neurons are active – only which brain areas are consuming the most energy, as shown by their oxygen consumption in real time. This limitation has motivated scientists to develop better techniques to visualise neuronal activity more directly. In photoacoustic tomography, this is done by revealing changing calcium concentrations within the neurons themselves.
Photoacoustic tomography using rodents promises to be a powerful tool, thanks to its 3D capabilities, its wide field of view and its high resolution (Ovsepian et al., 2017). In principle, it’s possible to image the whole brains of animals moving about freely as they find their way through a maze or solve other puzzles. Photoacoustic imaging currently lacks the resolution to record whole-brain function at the single-neuron level, but efforts are ongoing worldwide towards this goal.
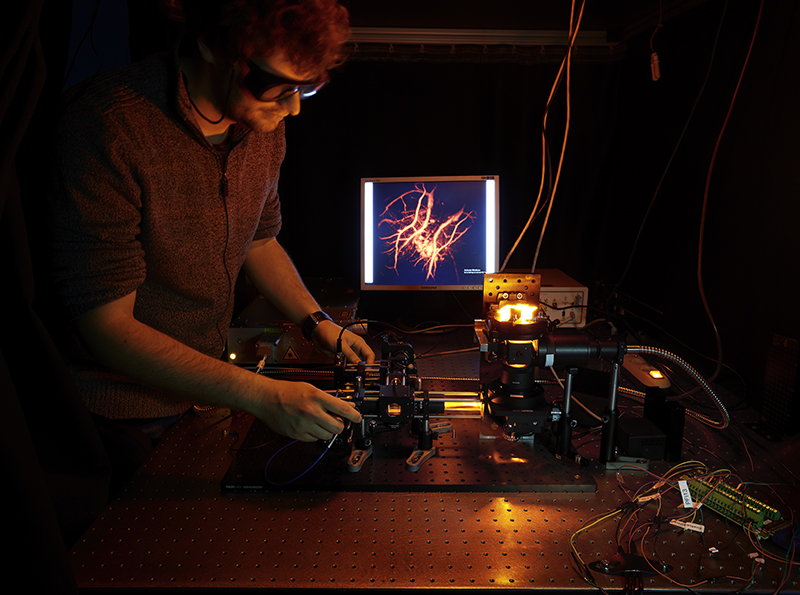
Marietta Schupp/EMBL
References
- Heijblom M et al. (2016) The state of the art in breast imaging using the Twente Photoacoustic Mammoscope: results from 31 measurements on malignancies. European Radiology 26: 3874-3887. doi: 10.1007/s00330-016-4240-7
- Jathoul AP et al. (2015) Deep in vivo photoacoustic imaging of mammalian tissues using a tyrosinase-based genetic reporter. Nature Photonics 9: 239-246. doi: 10.1038/nphoton.2015.22
- Ntziachristos V (2010) Going deeper than microscopy: the optical imaging frontier in biology. Nature Methods 7: 603-614. doi: 10.1038/nmeth.1483
- Ntziachristos V et al. (2005) Looking and listening to light: the evolution of whole-body photonic imaging. Nature Biotechnology 23: 313-320. doi: 10.1038/nbt1074
- Ovsepian S et al. (2017) Pushing the boundaries of neuroimaging with optoacoustics. Neuron 96: 966-988. doi: 10.1016/j.neuron.2017.10.022
Web References
- w1 – EMBL is Europe’s leading laboratory for basic research in molecular biology, with its headquarters in Heidelberg, Germany.
Resources
- Watch a video that explains the principles and applications of photoacoustic imaging.
Institutions
Review
This article shows how physics research can make a difference in the health sector – and gives the reader a sneak peak of imaging scans that will be available in a few years’ time.
The article could be used for project-based learning about different imaging technologies, and to make the topic of waves in physics seem more relevant to students. The link between biology and physics is also very interesting.
The article also supports teachers’ interest in innovative research, and the direct way it is written makes it easy for teachers to share the information with students – and perhaps inspire some to take up physics at tertiary level or beyond.
The article is suitable as a comprehension exercise. Suggested questions include:
- What happens to light when it passes through the body or biological tissue?
- Describe one advantage and one disadvantage of using X-ray imaging.
- Why are ultrasound images limiting for clinicians?
- What are chromophores?
- What is an advantage of photoacoustic imaging in microscopy?
Stephanie Maggi-Pulis, head of physics department, Secretariat for Catholic Education, Malta