The great migration Understand article
Why are cells like wildebeest? Laura Spinney investigates the migration of cells and the formation of organs, using the tiny and transparent zebrafish.
Every year in May, vast herds of wildebeest move from the dry East African plains towards the forests in search of food. They return in November, when the short rains come to water the plain, thus completing their annual migration. Each wildebeest behaves according to its instinct for survival, which tends to push it towards the company of other wildebeest. Once a herd has formed, it behaves as a new unit, with its own rules. Cells have a similar herd instinct. They are genetically programmed to work with other cells, but there is no strict set of instructions that determines the precise behaviour of every cell in the group. “We realise now that many of the events that occur after fertilisation are somehow free and plastic, and based on self-organising principles,” says Darren Gilmour from the European Molecular Biology Laboratory in Heidelberg, Germany.

Image courtesy of Darren Gilmour, EMBL
As a developmental biologist, Darren would like to understand those principles, because the mass migration of cells gives rise to the sculpting of intricate and complex systems such as blood vessels and the respiratory system. Since the cells within these migrating groups appear to be very similar in terms of the genes they express, knocking out (inactivating) or knocking down (reducing the activity of) single genes tends to block migration in the whole group. He has to take a more subtle approach, finding ways to disrupt the cells’ social networks, and looking at how that affects their ability to migrate and build organs. “It’s sociology, basically,” he says. “We want to know who tells who what to do, and how.”
Darren’s group explores these principles in the lateral line primordium of the zebrafish, a transient structure that can only be seen in early development, and whose function is to equip the embryonic fish with hair cell organs – sensory organs which enable it to detect the presence of other fish based on minute changes in water pressure. “The primordium is a cluster of more than 100 cells which creeps along under the skin of the animal, like a slug,” Darren explains. “It starts behind the ear, moving towards the tail, and as it goes it leaves a trail about two cells thick, punctuated by little clusters of cells that will eventually become hair cell organs.”
As model systems go, the zebrafish is an answer to biologists’ prayers, as it is transparent and therefore amenable to live imaging of cells which have been labelled with fluorescent proteins. The lateral line system is relatively easy to engineer genetically, and it is also a gift to those interested in cell migration, because it makes use of a signalling pathway that is very important in human health, and therefore well studied. A chemical signal called SDF1, the zebrafish equivalent of a signal also found in humans, causes a range of changes in the primordium cells. It does so by binding to a receptor, called Cxcr4b, that the cells carry on their exterior. Cxcr4b is a protein which sits across the cell membrane and, in humans, is necessary for HIV infection. As well as playing a role in normal development, it is also implicated in metastasis, or the spread of tumour cells.
In a zebrafish mutant with reduced Cxcr4b signalling, Darren’s group found that the primordium loses all directionality – there is no forward movement and no slug trail left behind. Moreover, the static structure seems to stop producing cell clusters, as if it has to move and expel those clusters in order to generate more. One of the most interesting features of the mutant, however, is that even though the primordium’s migration is stalled, a closer look reveals that its cells are still moving, tumbling over one another in an apparently random fashion.w1
To find out if they could rescue the mutant and restore forward motion to the primordium, the researchers created a genetic mosaic by transplanting some normal, ‘seeing’ cells, which expressed the Cxcr4b receptor and could therefore respond to SDF1a, into the otherwise ‘blind’ mutant tissue. The normal and mutant cells were tagged with red and green fluorescent proteins respectively, so that Darren and his team could track the cells’ movements. Very quickly, through the tumbling motion of the cells in the primordium, the seeing cells found their way to the front, and the primordium once again moved forward.
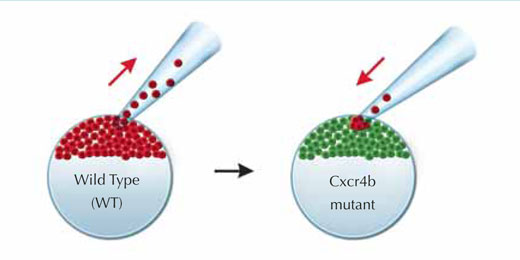
Image courtesy of Petra Riedinger, EMBL
What was strange about these experiments, says Darren, was that not all of the sensing, Cxcr4b-expressing cells found their way to the front. As soon as some were there, the random tumbling motion stopped, the primordium moved forward, and those sensing cells that had not made it to the front remained where they were –“as if frozen in ice”. “We think that the system finds strength in instability,” he says. “It rolls around and makes random movements, and in doing so it ensures that the cells that can lead get into the position where they do lead. Whenever they pull, the others freeze.”
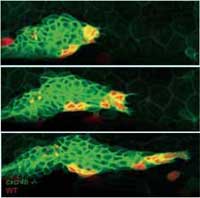
cells (red) find their way to the
tip of the primordium and
project in the direction of
migration, pulling mutant cells
(green) with them.
Click to enlarge image
Image courtesy of Darren Gilmour,
EMBL
But if not all sensing cells become leaders, what defines a leader? Whatever it is, it doesn’t appear to be differences in the activity of genes. “It’s not the genes that say, you’re the leader, you’re the follower,” Darren says. “Rather the genes equip all the cells with the receptor, and after that it’s a case of ‘fight amongst yourselves’.” He and his co-worker Petra Haas think leadership is more likely to be defined by the unstable nature of the migrating group. The random tumbling of the cells gives some a temporary bias over others, in terms of their proximity to the source of the signal. They then latch on to it, and if they are able to maintain the activation of their receptor, they move to the front. However, that is not the end of the story, by any means.
One theory of cell migration is that the leading cells somehow carry the followers, which passively allow themselves to be swept along. However Darren is sceptical of what he calls the hitchhiker model. He prefers to think that the leaders point the others in the right direction, and the primordium is internally organised such that those others then actively follow. And he believes that a similar principle will apply to the development of all organs. “If you look at the branching of blood vessels, I predict that there will be a small number of cells at the tip which sense the signals from the environment, and through cell-cell interactions they somehow influence the behaviour of the others,” he says. “The others don’t have to see the world.” He gives the analogy of a falcon ambushing a flock of starlings. Not all the starlings have to see the falcon in order to respond appropriately by fleeing – they only have to detect the panicked response of their neighbours.
So far so good, but the story gets more complicated still, because it turns out that not all the followers behave in the same way. In their live-imaging experiments, Darren and his team have noticed that as the front of the primordium noses forward, the cells in the rear slow down, causing the tissue to stretch. PhD student Guillaume Valentin has now identified another receptor, expressed by those rear cells, which also responds to SDF1a, but which triggers a different kind of behaviour to that elicited by the SDF1a/Cxcr4b pathway – giving the cells at the back a certain degree of independence from the Cxcr4b-expressing leader cells.
When the primordium is moving forwards, depositing cell clusters at the back, the general motion of cells through the structure is backwards. As cells fall back inside it, Darren suspects they may switch on this second receptor. Perhaps, he speculates, the combination of the two cell populations expressing different receptors gives rise to a kind of caterpillar motion, in which the front and back of the primordium move forward alternately. Some evidence for this comes from experiments with zebrafish embryos in which the activity of the second receptor is reduced and the second half of that coordinated movement is lacking. “The leading edge pulls and pulls, but the back doesn’t come with it, so eventually it gives up and tumbles back,” he says.
If he is correct, the new findings raise questions about how dynamic information is transmitted through large groups of cells. It becomes necessary to understand not only signalling, but also the propagation of forces through cell populations, in terms of cell-cell interactions. This is where Darren now hopes to take his research. For now, though, he is excited by the discovery of a second SDF1a receptor in the zebrafish primordium. “The idea that the cells inside can also sense is entirely novel,” he says. Whether the same principle applies to wildebeest is another matter.
Resources
- Haas P, Gilmour D (2006) Chemokine signaling mediates self-organizing tissue migration in the zebrafish lateral line. Developmental Cell 10: 673-680. doi: 10.1016/j.devcel.2006.02.019
Institutions
Review
I was delighted to be asked to review this paper, as I might not have read it so carefully otherwise. It is so well written, with many colourful analogies, such as linking the migration of African herd animals with the migration of cells in developing organisms. Not only is the biology fascinating, including signal proteins, HIV infection, zebra fish, cancer cells and mutant ‘blind’ cells, but so is the description of the research team’s methodology, which reads like a detective story: coming up with theories, rejecting models, moving onto new ideas and testing them.
There is a lot of interest in this article for most biology teachers. It could also make a really good comprehension exercise or provide supplementary material for biology students aged 16 or more. Alternatively, it could be used in a discussion of embryology, vertebrate development, genetic engineering, communication between cells as opposed to communication between organisms, signal proteins, cancer, mutations, the steps in scientific investigations or modelling theories.
Sue Howarth, UK